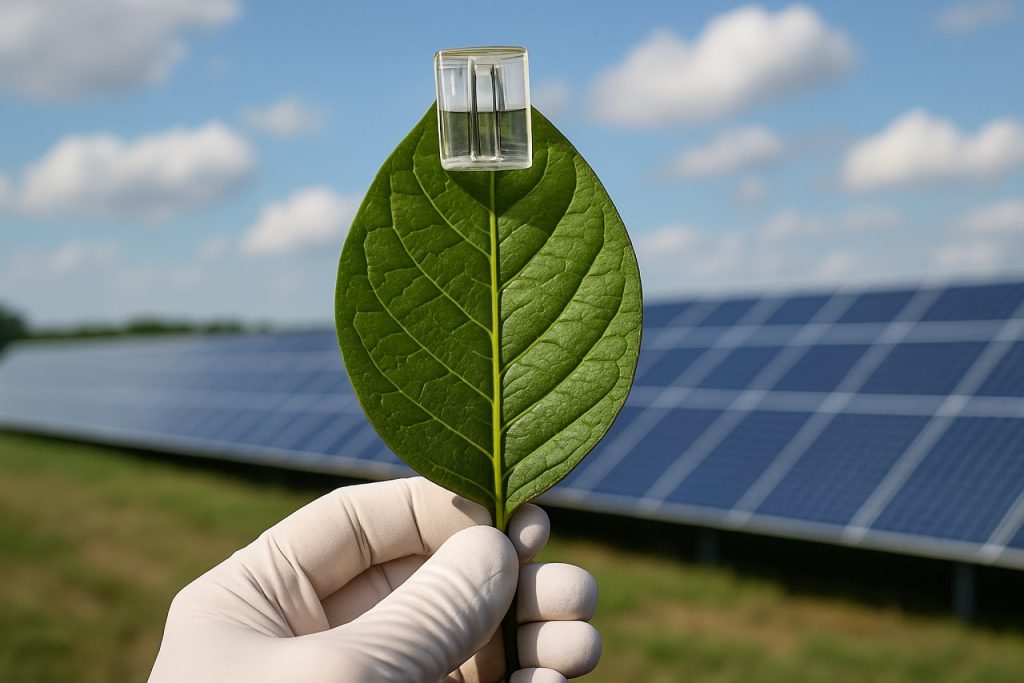
Artificial Leaf Technology Explained: How Bio-Inspired Innovation is Transforming Solar Fuel Production and the Future of Sustainable Energy. Discover the Science, Breakthroughs, and Global Impact. (2025)
- Introduction: The Concept and Promise of Artificial Leaf Technology
- Historical Development and Key Scientific Breakthroughs
- How Artificial Leaves Mimic Photosynthesis: Core Mechanisms
- Leading Research Institutions and Industry Players
- Materials, Design, and Engineering Challenges
- Current Applications: From Hydrogen Production to Carbon Capture
- Market Growth and Public Interest: 2024–2030 Forecasts
- Environmental Impact and Sustainability Assessment
- Policy, Regulation, and Funding Landscape
- Future Outlook: Scaling, Commercialization, and Global Adoption
- Sources & References
Introduction: The Concept and Promise of Artificial Leaf Technology
Artificial leaf technology represents a groundbreaking approach to sustainable energy and carbon management, inspired by the natural process of photosynthesis. The core concept involves engineered devices that mimic the ability of plant leaves to capture sunlight and convert carbon dioxide (CO2) and water into energy-rich compounds. Unlike traditional solar panels, artificial leaves aim not only to generate electricity but also to produce fuels or valuable chemicals directly from atmospheric CO2, offering a dual benefit of renewable energy production and carbon capture.
The promise of artificial leaf technology has gained significant momentum in recent years, with research institutions and organizations worldwide accelerating development. Notably, the University of Cambridge has pioneered artificial leaf prototypes capable of converting sunlight, water, and CO2 into syngas—a mixture of hydrogen and carbon monoxide that can be further processed into liquid fuels. Their 2022 demonstration of a standalone device operating under real-world conditions marked a critical step toward practical deployment.
In parallel, the California Institute of Technology (Caltech) and the Jet Propulsion Laboratory have advanced the field through the Joint Center for Artificial Photosynthesis, focusing on scalable systems for solar-driven fuel production. These efforts are complemented by the Helmholtz Association in Germany, which is exploring integrated artificial leaf platforms for industrial applications.
The urgency of climate change and the global push for net-zero emissions by 2050 have intensified interest in artificial leaf technology. According to the International Energy Agency, direct air capture and utilization technologies, including artificial leaves, are expected to play a growing role in decarbonizing sectors that are difficult to electrify. As of 2025, several pilot projects are underway, with the next few years likely to see increased investment and the first commercial-scale demonstrations.
Looking ahead, artificial leaf technology holds the potential to transform the energy landscape by enabling decentralized, carbon-neutral fuel production. If technical challenges—such as efficiency, durability, and cost—are addressed, artificial leaves could become a cornerstone of future clean energy systems, supporting both climate mitigation and energy access goals.
Historical Development and Key Scientific Breakthroughs
Artificial leaf technology, inspired by natural photosynthesis, has evolved significantly since its conceptual inception in the late 20th century. The core idea is to mimic the process by which plants convert sunlight, water, and carbon dioxide into energy-rich compounds, but to do so with engineered materials and systems for sustainable fuel production. The journey from early laboratory prototypes to the cusp of commercial viability in 2025 has been marked by several pivotal breakthroughs and the involvement of leading research institutions and organizations.
The first major milestone was achieved in 2011, when researchers at Harvard University—notably Dr. Daniel Nocera—demonstrated a practical artificial leaf device capable of splitting water into hydrogen and oxygen using sunlight and earth-abundant catalysts. This innovation addressed the challenge of using non-precious metals, making the technology more scalable and affordable. The device’s ability to operate in simple water, even if impure, was a significant step toward real-world application.
Subsequent years saw rapid progress in catalyst efficiency, stability, and selectivity. By the early 2020s, teams at institutions such as University of Cambridge and California Institute of Technology had developed artificial leaves that not only split water but also directly reduced carbon dioxide into energy-dense fuels like syngas and formic acid. In 2019, Cambridge researchers unveiled a device that could convert sunlight, CO2, and water into syngas—a precursor for liquid fuels—without producing unwanted byproducts, marking a leap toward carbon-neutral fuel cycles.
The period from 2020 to 2025 has been characterized by a shift from proof-of-concept devices to scalable prototypes and pilot projects. In 2022, the University of Cambridge announced a floating artificial leaf system capable of operating on open water, broadening the potential deployment scenarios for the technology. Meanwhile, collaborative efforts between academic labs and industry, such as those supported by the U.S. Department of Energy, have focused on integrating artificial leaf modules into existing energy infrastructures and improving their efficiency under real-world conditions.
As of 2025, artificial leaf technology stands at a critical juncture. The most advanced systems now achieve solar-to-fuel conversion efficiencies approaching 10%, with ongoing research targeting the 15% threshold considered necessary for commercial competitiveness. The next few years are expected to see increased investment in pilot-scale demonstrations, particularly in regions with abundant sunlight and water resources. The outlook is cautiously optimistic: if current trends in materials science and systems engineering continue, artificial leaf technology could play a transformative role in the global transition to sustainable fuels by the late 2020s.
How Artificial Leaves Mimic Photosynthesis: Core Mechanisms
Artificial leaf technology represents a cutting-edge approach to sustainable energy, aiming to replicate the natural process of photosynthesis to convert sunlight, water, and carbon dioxide into usable fuels. The core mechanisms of artificial leaves are inspired by the way plants harness solar energy, but they employ advanced materials and engineered systems to achieve higher efficiencies and broader applications.
At the heart of artificial leaf systems is the photoelectrochemical (PEC) cell, which typically consists of semiconductor materials that absorb sunlight and generate electron-hole pairs. These charge carriers drive redox reactions at the leaf’s surface, splitting water molecules into hydrogen and oxygen, or reducing carbon dioxide into energy-rich hydrocarbons. Recent prototypes, such as those developed by University of Cambridge researchers, utilize perovskite-based semiconductors and molecular catalysts to enhance light absorption and catalytic efficiency. Their 2023 demonstration of a wireless artificial leaf floating on water showcased the direct conversion of sunlight and water into syngas—a mixture of hydrogen and carbon monoxide—without external wires or power sources.
A key innovation in current artificial leaf designs is the integration of selective catalysts that mimic the role of natural enzymes. For example, cobalt-phosphate and nickel-based catalysts are used to facilitate the oxygen evolution reaction, while copper or silver catalysts are employed for carbon dioxide reduction. These materials are chosen for their abundance, stability, and ability to operate under ambient conditions, making them suitable for scalable deployment. The National Renewable Energy Laboratory (NREL) in the United States is actively researching new catalyst compositions and nanostructures to further improve conversion efficiencies and operational lifetimes.
Another critical aspect is the membrane or separator that prevents the recombination of generated gases, ensuring safe and efficient collection. Advanced ion-exchange membranes, inspired by plant cell walls, are being developed to optimize ion transport while maintaining product purity. The Helmholtz Association in Germany, a major research organization, is leading efforts to design robust membranes for artificial photosynthesis devices.
Looking ahead to 2025 and beyond, artificial leaf technology is expected to transition from laboratory-scale demonstrations to pilot-scale outdoor testing. The focus will be on increasing solar-to-fuel conversion efficiencies, reducing material costs, and integrating artificial leaves into decentralized energy systems. With ongoing support from major research institutions and government initiatives, artificial leaves could play a pivotal role in the global shift toward carbon-neutral fuels and distributed renewable energy production within the next few years.
Leading Research Institutions and Industry Players
Artificial leaf technology, which seeks to mimic natural photosynthesis for sustainable fuel and chemical production, has seen significant advancements in recent years. As of 2025, several leading research institutions and industry players are at the forefront of this field, driving innovation and moving towards commercialization.
Among academic institutions, the Harvard University research group led by Professor Daniel Nocera has been a pioneer. Their work on the “bionic leaf” has demonstrated the conversion of sunlight, water, and air into liquid fuels and fertilizers, with ongoing efforts to improve efficiency and scalability. Similarly, the University of Cambridge has made notable progress, particularly through the team led by Professor Erwin Reisner, which has developed artificial leaves capable of producing syngas directly from sunlight and carbon dioxide. These breakthroughs have been published in peer-reviewed journals and are frequently cited as benchmarks in the field.
In Asia, the Tsinghua University in China is recognized for its research on photoelectrochemical cells and catalyst development for artificial photosynthesis. Their collaborations with national laboratories and industry partners are accelerating the translation of laboratory results into pilot-scale demonstrations.
On the industry side, several companies are emerging as key players. Siemens, a global engineering conglomerate, has invested in artificial photosynthesis as part of its broader decarbonization strategy, focusing on integrating artificial leaf modules with renewable energy systems. Shell has also announced research partnerships with academic groups to explore artificial leaf technology for sustainable fuel production, aligning with its long-term net-zero ambitions.
Government-backed organizations are playing a crucial role as well. The U.S. Department of Energy (DOE) funds multiple artificial photosynthesis projects through its Joint Center for Artificial Photosynthesis (JCAP), a consortium that includes the California Institute of Technology and Lawrence Berkeley National Laboratory. JCAP’s mission is to develop scalable, efficient systems for solar fuel generation, and its research outputs are shaping the direction of the field.
Looking ahead, the next few years are expected to see increased collaboration between academia and industry, with pilot projects and demonstration plants anticipated by 2027. The convergence of advanced materials, catalysis, and systems engineering is likely to accelerate the path toward commercial viability, positioning artificial leaf technology as a promising contributor to the global clean energy transition.
Materials, Design, and Engineering Challenges
Artificial leaf technology, inspired by natural photosynthesis, aims to convert sunlight, water, and carbon dioxide into fuels or valuable chemicals. As of 2025, the field is advancing rapidly, but significant materials, design, and engineering challenges remain before large-scale deployment is feasible.
A core challenge lies in the selection and optimization of photoactive materials. Artificial leaves typically use semiconductors such as silicon, titanium dioxide, or perovskites to absorb sunlight and drive chemical reactions. However, these materials must balance efficiency, stability, and cost. For example, while silicon is abundant and well-understood, it is prone to corrosion in aqueous environments, limiting its operational lifespan. Researchers at institutions like California Institute of Technology and University of Cambridge are developing protective coatings and hybrid structures to enhance durability and performance.
Catalyst design is another critical area. Artificial leaves require catalysts to facilitate the reduction of CO2 or the splitting of water into hydrogen and oxygen. Noble metals such as platinum and iridium are highly effective but expensive and scarce. Recent efforts focus on earth-abundant alternatives, such as nickel, cobalt, or molecular catalysts based on organic frameworks. For instance, the Japan Science and Technology Agency is supporting projects to develop robust, low-cost catalysts that maintain high activity and selectivity under real-world conditions.
Engineering challenges also include integrating all components—light absorbers, catalysts, and membranes—into a single, scalable device. Achieving efficient charge separation and minimizing energy losses at interfaces are ongoing concerns. The design must also ensure that the artificial leaf operates safely and efficiently in variable outdoor environments, handling fluctuations in sunlight, temperature, and humidity. The National Renewable Energy Laboratory in the United States is actively researching device architectures that maximize surface area and light capture while maintaining structural integrity.
Looking ahead, the next few years are expected to see progress in the development of modular, scalable prototypes and pilot demonstrations. Collaborative efforts between academic institutions, government agencies, and industry partners are accelerating the translation of laboratory breakthroughs into practical systems. However, widespread adoption will depend on further advances in materials durability, cost reduction, and system integration. The outlook for artificial leaf technology in 2025 and beyond is promising, but overcoming these engineering and materials challenges remains essential for commercial viability.
Current Applications: From Hydrogen Production to Carbon Capture
Artificial leaf technology, inspired by natural photosynthesis, has rapidly advanced from laboratory prototypes to early-stage real-world applications, particularly in hydrogen production and carbon capture. As of 2025, several research institutions and companies are demonstrating artificial leaves that use sunlight to split water into hydrogen and oxygen, or to convert atmospheric carbon dioxide into useful fuels and chemicals.
One of the most prominent applications is solar-driven hydrogen production. Artificial leaves typically employ photoelectrochemical (PEC) cells, where semiconductor materials absorb sunlight and catalyze water splitting. The University of Cambridge has developed a floating artificial leaf that can generate hydrogen fuel from water sources, including rivers and lakes, without relying on purified water or external electricity. Their device uses perovskite-based light absorbers and earth-abundant catalysts, achieving solar-to-hydrogen efficiencies above 1%, with ongoing efforts to scale up and improve stability.
In the United States, the National Renewable Energy Laboratory (NREL) is actively researching PEC water splitting, targeting cost-effective and durable materials for large-scale hydrogen production. NREL’s recent prototypes have demonstrated stable operation over hundreds of hours, a key milestone for commercial viability. The U.S. Department of Energy’s Hydrogen Shot initiative, which aims to reduce the cost of clean hydrogen to $1 per kilogram within a decade, is expected to accelerate artificial leaf deployment in the coming years.
Beyond hydrogen, artificial leaves are being engineered for direct carbon capture and conversion. The California Institute of Technology (Caltech) and its Joint Center for Artificial Photosynthesis have created devices that reduce atmospheric CO2 into carbon monoxide or formic acid, which can serve as building blocks for synthetic fuels. These systems integrate CO2-absorbing membranes with light-activated catalysts, and recent prototypes have achieved selectivity rates above 90% for targeted products.
Looking ahead, the next few years are expected to see pilot-scale demonstrations and field trials of artificial leaf systems, particularly in regions with abundant sunlight and water resources. Key challenges remain, including improving device durability, reducing costs, and integrating artificial leaves with existing energy and chemical infrastructure. However, with continued investment from government agencies and collaborations with industry, artificial leaf technology is poised to play a significant role in the global transition to sustainable hydrogen and carbon-neutral fuels.
Market Growth and Public Interest: 2024–2030 Forecasts
Artificial leaf technology, which mimics natural photosynthesis to convert sunlight, water, and carbon dioxide into fuels or valuable chemicals, is gaining momentum as a promising solution for sustainable energy and carbon capture. As of 2025, the market for artificial leaf systems remains in its early stages, with most developments concentrated in research institutions and pilot-scale demonstrations. However, the next few years are expected to see significant advancements, driven by increasing public and governmental interest in climate mitigation and renewable energy innovation.
Key players in the field include leading academic institutions and research organizations such as University of Cambridge, which has developed artificial leaves capable of producing syngas directly from sunlight and air, and California Institute of Technology, home to the Joint Center for Artificial Photosynthesis (JCAP), a major U.S. initiative focused on scalable solar fuel production. These organizations are collaborating with industry partners to move from laboratory prototypes to scalable, commercially viable systems.
Recent breakthroughs have improved the efficiency and stability of artificial leaf devices. For example, in 2024, researchers at the University of Cambridge reported a device that can operate in real-world conditions, using only sunlight and air to produce liquid fuels. Such advances are expected to accelerate technology transfer and attract investment from both public and private sectors.
Governmental support is also increasing. The European Union’s Horizon Europe program and the U.S. Department of Energy are funding projects aimed at scaling up artificial photosynthesis technologies. These initiatives reflect a broader policy trend toward decarbonization and the development of negative emissions technologies, which is likely to boost market growth through 2030.
Forecasts for the artificial leaf market suggest a compound annual growth rate (CAGR) in the double digits, although precise figures remain speculative due to the nascent stage of commercialization. The main drivers include the urgent need for carbon-neutral fuels, the global push for net-zero emissions, and the versatility of artificial leaf systems in producing hydrogen, methanol, and other chemicals directly from atmospheric CO2.
Public interest is expected to rise as demonstration projects and pilot plants become more visible, especially in regions with strong climate policies. Educational outreach by organizations such as California Institute of Technology and University of Cambridge is also helping to raise awareness of the technology’s potential. By 2030, artificial leaf technology could transition from a research-driven field to an emerging market segment within the broader clean energy landscape, provided that current technical and economic challenges are addressed.
Environmental Impact and Sustainability Assessment
Artificial leaf technology, inspired by natural photosynthesis, is rapidly advancing as a promising solution for sustainable fuel and chemical production. In 2025, the environmental impact and sustainability of artificial leaves are under close scrutiny as research transitions from laboratory prototypes to pilot-scale demonstrations. The core principle involves using sunlight to convert water and carbon dioxide into energy-rich compounds, such as hydrogen or carbon-based fuels, with minimal emissions.
Recent developments have focused on improving the efficiency and durability of artificial leaf systems. For example, researchers at the University of Cambridge have demonstrated artificial leaves capable of converting sunlight, water, and CO2 into syngas—a precursor for sustainable liquid fuels—without relying on fossil resources. These systems operate under ambient conditions and use earth-abundant materials, reducing the environmental footprint compared to traditional chemical processes.
A key sustainability advantage of artificial leaf technology is its potential to close the carbon loop. By capturing atmospheric or industrial CO2 and converting it into usable fuels, artificial leaves can help mitigate greenhouse gas emissions. The Helmholtz Association, a major German research organization, is actively investigating the life cycle impacts of artificial photosynthesis, emphasizing the importance of renewable energy inputs and the recyclability of catalyst materials.
Water use is another critical factor in sustainability assessments. Artificial leaves typically require only modest amounts of water, and ongoing research aims to enable operation with non-potable or saline water sources, further reducing competition with agricultural or drinking water supplies. The National Renewable Energy Laboratory (NREL) in the United States is evaluating the integration of artificial leaf systems with existing water infrastructure to maximize resource efficiency.
Looking ahead, the scalability and deployment of artificial leaf technology will determine its true environmental impact. Pilot projects in Europe and Asia are expected to provide real-world data on energy payback times, land use, and emissions profiles over the next few years. If current efficiency gains continue, artificial leaves could play a significant role in decarbonizing sectors such as transportation and chemical manufacturing by 2030.
In summary, artificial leaf technology in 2025 is positioned as a sustainable alternative for renewable fuel production, with ongoing research addressing challenges related to material sustainability, water use, and system integration. The next few years will be critical for validating its environmental benefits at scale and establishing its role in global climate strategies.
Policy, Regulation, and Funding Landscape
Artificial leaf technology, which mimics natural photosynthesis to convert sunlight, water, and carbon dioxide into fuels or chemicals, is gaining increasing attention from policymakers and funding agencies as part of broader decarbonization and renewable energy strategies. In 2025, the policy and regulatory landscape is characterized by a mix of targeted research funding, early-stage demonstration support, and integration into national and international clean energy agendas.
The European Union continues to be a significant driver, with the European Commission supporting artificial photosynthesis through its Horizon Europe program. The European Commission has identified solar fuels and artificial photosynthesis as key enabling technologies for the EU’s 2050 climate neutrality goal, with dedicated calls for projects under the “Clean Energy Transition” cluster. The Helmholtz Association in Germany, one of Europe’s largest scientific organizations, is coordinating multi-institute efforts to advance artificial leaf prototypes and scale-up, supported by both national and EU-level funding.
In the United States, the U.S. Department of Energy (DOE) continues to fund artificial photosynthesis research through its Solar Energy Technologies Office and the Fuels from Sunlight program. The DOE’s Energy Earthshots Initiative, launched in 2021, includes the “Hydrogen Shot,” which aims to reduce the cost of clean hydrogen production, with artificial leaf technology considered a potential contributor. The DOE is expected to announce new funding opportunities in 2025 for pilot-scale demonstrations of solar-to-fuel systems, building on progress from the Joint Center for Artificial Photosynthesis (JCAP), a leading U.S. research consortium.
In Asia, Japan’s New Energy and Industrial Technology Development Organization (NEDO) and South Korea’s Korea Institute of Science and Technology (KIST) are investing in artificial leaf research as part of their national hydrogen and carbon-neutrality strategies. These agencies are supporting both academic and industrial consortia to accelerate the translation of laboratory breakthroughs into scalable prototypes.
On the regulatory front, artificial leaf technology is not yet subject to specific standards or permitting frameworks, but is increasingly referenced in national hydrogen roadmaps and low-carbon fuel standards. The next few years are likely to see the emergence of guidelines for safety, environmental impact, and integration with existing energy infrastructure, especially as pilot projects move toward commercialization.
Overall, the funding and policy environment in 2025 is supportive but still focused on research, development, and demonstration. The outlook for the next few years includes increased public investment, the first large-scale demonstration plants, and the gradual development of regulatory frameworks as artificial leaf technology moves closer to market readiness.
Future Outlook: Scaling, Commercialization, and Global Adoption
Artificial leaf technology, which mimics natural photosynthesis to convert sunlight, water, and carbon dioxide into fuels or valuable chemicals, is poised for significant developments in 2025 and the following years. The field has moved from laboratory-scale demonstrations to early-stage pilot projects, with a focus on scaling, commercialization, and global adoption.
In 2025, several leading research institutions and companies are expected to advance their artificial leaf prototypes toward larger-scale field trials. For example, University of Cambridge researchers, who have pioneered solar-driven CO2 conversion devices, are working on integrating their systems into modular units suitable for decentralized fuel production. Their recent breakthroughs in using perovskite-based photoelectrodes have improved efficiency and stability, key factors for commercial viability.
On the industrial front, Shell and other major energy companies have signaled interest in artificial photosynthesis as part of their broader decarbonization strategies. These organizations are exploring partnerships with academic groups and startups to accelerate the transition from proof-of-concept to pilot-scale demonstrations. The goal is to produce sustainable fuels such as hydrogen or syngas directly from sunlight and air, bypassing fossil resources.
Governmental and international organizations are also playing a crucial role. The U.S. Department of Energy continues to fund artificial photosynthesis research through initiatives like the Liquid Sunlight Alliance, aiming to develop scalable, integrated systems. Similarly, the European Commission supports projects under its Horizon Europe program, targeting the deployment of artificial leaf technologies for industrial and transportation sectors.
Despite these advances, several challenges remain for widespread adoption. Key hurdles include improving device durability, reducing costs of catalyst materials, and integrating artificial leaf systems with existing energy infrastructure. Researchers are optimistic that, by 2027, pilot plants capable of producing liters of fuel per day will be operational, providing critical data for techno-economic analysis and life-cycle assessment.
Looking ahead, the global outlook for artificial leaf technology is cautiously optimistic. If current progress continues, the late 2020s could see the first commercial deployments in regions with abundant sunlight and supportive policy frameworks. Success will depend on continued collaboration between academia, industry, and government, as well as public investment to bridge the gap between laboratory innovation and market-ready solutions.
Sources & References
- University of Cambridge
- California Institute of Technology
- Helmholtz Association
- International Energy Agency
- Harvard University
- National Renewable Energy Laboratory
- Tsinghua University
- Siemens
- Shell
- Japan Science and Technology Agency
- European Commission
- New Energy and Industrial Technology Development Organization